ADVERTISEMENT
Intravascular Imaging for Infra-Inguinal Peripheral Artery Disease Intervention
A Review of Current Methods and Existing Data
A Review of Current Methods and Existing Data
© 2023 HMP Global. All Rights Reserved.
Any views and opinions expressed are those of the author(s) and/or participants and do not necessarily reflect the views, policy, or position of Vascular Disease Management or HMP Global, their employees, and affiliates.
VASCULAR DISEASE MANAGEMENT. 2023;20(7):E130-E139
Abstract
Symptomatic infrainguinal peripheral artery disease affects ≥100 million individuals worldwide and is increasingly being managed with endovascular intervention. Many experts recognize that the success of peripheral endovascular treatment is heavily dependent on the data provided by the imaging modality used during the case. While angiography has traditionally been the gold standard, research supporting the use of other forms of intravascular imaging (intravascular ultrasound [IVUS] and optical coherence tomography [OCT]) has been emerging, with the use of IVUS now being recommended in all phases of infrainguinal intervention. This manuscript reviews the applications and supporting data for the use of IVUS and OCT in infrainguinal endovascular intervention.
Background
Lower extremity peripheral artery disease (PAD) affects more than 235 million individuals worldwide and is a leading cause of cardiovascular morbidity and mortality.1 Nearly half of patients with lower extremity PAD experience some form of exertional claudication, rest pain, or tissue loss resulting from reduction in blood flow and seek treatment for their condition.2 While many are still managed with open surgical bypass, the field of endovascular intervention has expanded over the past 20 years and is able to offer minimally invasive treatment options to patients who are often poor surgical candidates.
Many experts recognize that the success of peripheral endovascular treatment is heavily dependent on the data provided by the imaging modality used during the case. While angiography has traditionally been the gold standard, its characterization of the vessel and its borders is limited by the fact that it only provides a 2-dimensional (2D) representation of a 3-dimensional (3D) object. In addition, the use of angiography entails radiation and contrast exposure, which can be harmful to a population of patients with a high frequency of comorbid renal insufficiency. In recognition of these limitations, the use of other forms of intravascular imaging, including intravascular ultrasound (IVUS) and optical coherence tomography (OCT), has been increasing.3 These imaging modalities have been shown to optimize periprocedural and postprocedural outcomes in the coronary vasculature, and many operators are optimistic that these benefits will extend to the peripheral circulation as well.4-7 The aim of this manuscript is to review the applications and supporting literature for IVUS and OCT use in infrainguinal endovascular intervention.
Intravascular Ultrasound (IVUS)
IVUS functions using an ultrasound transducer mounted on the tip of a catheter which, when delivered into the target vessel, provides a 360-degree view of the vessel lumen and vessel wall. The applications and data supporting IVUS use pre-, intra-, and post-infrainguinal intervention are described below.
Preintervention
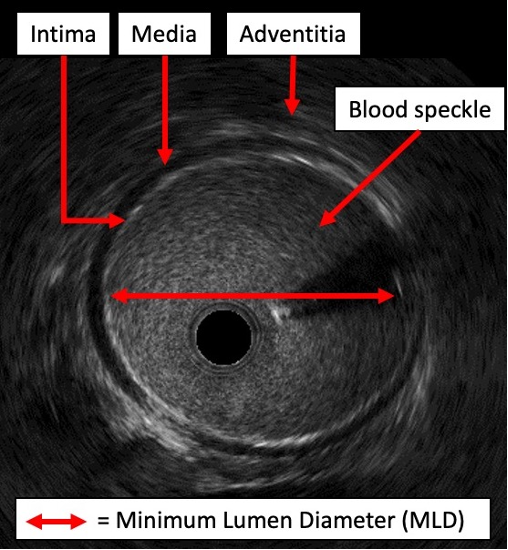
Prior to infrainguinal intervention, IVUS can be used to generate precise measurements of the target vessel lumen. On IVUS imaging, the borders of the vessel wall are clearly demarcated, with the media appearing dark and echolucent and the surrounding intima (internally) and adventitia (externally) appearing bright and echogenic (Figure 1). This enables the operator to make precise measurements of the diseased lumen (diameter, cross-sectional area) as well as the reference vessel diameter (RVD). Several studies in the infrainguinal circulation suggest that IVUS provides more precise and substantially larger vessel measurements than angiography. In a prospective study of 1725 patients with symptomatic femoropopliteal PAD, Iida et al demonstrated that IVUS-assessed RVD was significantly larger than angiography-assessed RVD by almost 1 mm (6 mm vs 5 mm, P<.001), with 49% of limbs in the study population having RVD discrepancies of ≥1 mm.8 Pliagas et al demonstrated similar discrepancies in a retrospective study of 43 patients with infrainguinal PAD, demonstrating that IVUS estimates were on average 0.6 mm to 1.3 mm larger than angiography-derived estimates.9 The magnitude of these differences relative to the vessel size is amplified in the infrapopliteal circulation. In a population of 20 patients with infrapopliteal PAD, Shammas et al demonstrated that quantitative vascular angiography measurements were on average 1.1 mm smaller than IVUS-derived measurements for the same treated vessel (2.9 mm vs 4.0 mm, P<.001)10. This equated to a size discrepancy of almost 38%. Kuku et al demonstrated a similar finding during a calibration exercise for the LIFE-BTK study, with angiography yielding RVD estimates 0.5 mm smaller on average than IVUS estimates (2.8 ± 1.2 mm vs 3.3 ± 0.7 mm, respectively).11
Overall, the existing literature in the infrainguinal circulation suggests that angiography significantly underestimates RVD, which has the potential to impact both treatment decisions and outcomes.
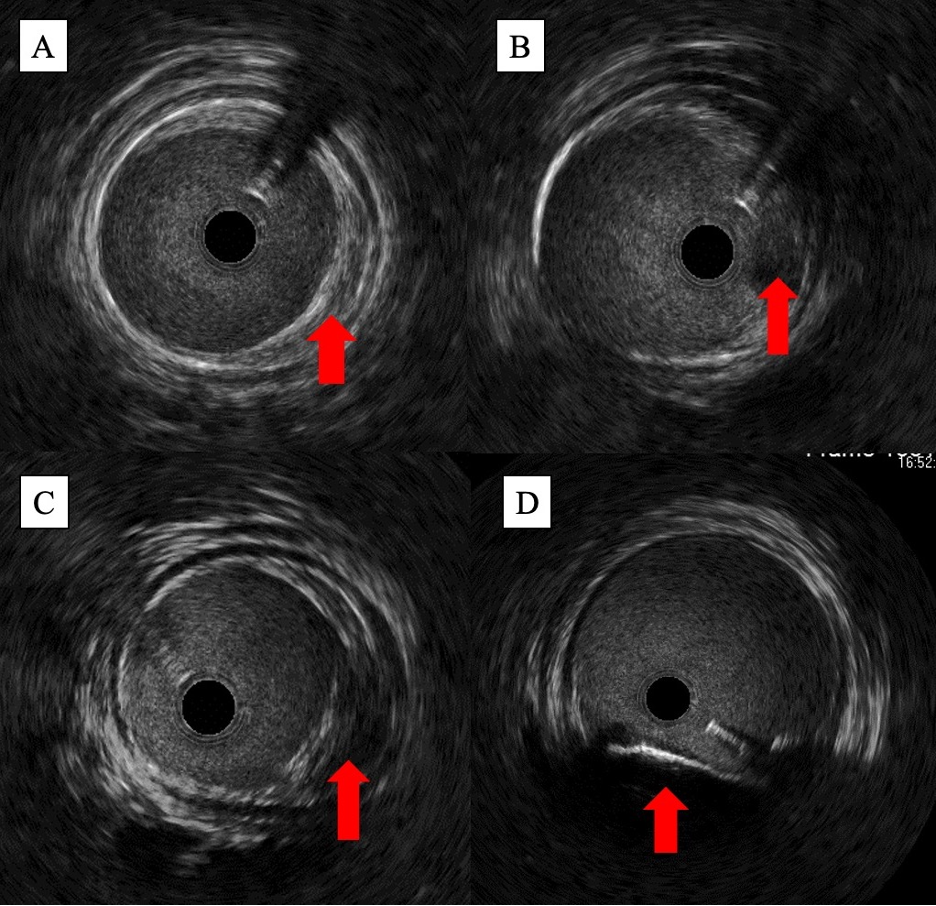
In addition to providing more accurate luminal measurements, IVUS enables a more detailed characterization of plaque composition. With IVUS, operators can classify plaque composition into 1 of 4 categories: fibrous, fatty, fibro-fatty, or calcific (Figure 2).12 On the grayscale image, fatty plaque appears echolucent, while fibrous and calcific plaques appear echogenic. Calcific plaque can be distinguished from fibrous plaque by the presence of posterior acoustic shadowing. Several studies support that IVUS has superior sensitivity over angiography in identifying calcified plaque in the infrainguinal vessels. In a subanalysis of 47 patients with femoropopliteal PAD and severe superficial calcification in the JetStream G3 calcium study, Yin et al demonstrated through independent core lab analysis that IVUS was able to detect calcium in a significantly higher percentage of lesions than angiography (93.6% of the study population vs 55.3%, P<.0001).13 This correlated with a sensitivity and specificity of 59% and 100% when angiography was compared with IVUS for calcium identification. Given its superior ability to identify calcium, IVUS has been utilized to validate several angiographic calcium scoring systems, including Peripheral Academic Research Consortium (PARC), Peripheral Arterial Calcium Score System (PACSS), and DEFINITIVE Ca++.13
By accurately delineating plaque composition, IVUS can assist operators in determining if vessel preparation (atherectomy or lithotripsy) is needed prior to angioplasty or stenting. In the JETSTREAM, TRUE, and TRUTH studies, which investigated the use of different atherectomy devices in infrainguinal plaque modification, IVUS was the primary technique utilized to identify plaque morphology and volume prior to and immediately following atherectomy.14-16 In addition to identifying vessels that will need plaque modification, IVUS can also help operators determine how plaque modification is progressing; in a study of 40 patients undergoing atherectomy for symptomatic femoropopliteal PAD, Tielbeek et al demonstrated that IVUS assessment prompted additional passes of the atherectomy device in 15 of the 18 patients who underwent IVUS assessment in addition to digital subtraction angiography.17 These additional passes resulted in significantly greater lumen gain for the IVUS-guided group.
By providing a 360-degree view of the vessel wall, IVUS can also delineate plaque morphology in a way that 2D angiographic images cannot. On the IVUS image, concentric plaque can be differentiated from eccentric plaque based on the presence or absence of circumferential wall involvement. If the plaque is calcific, operators can also quantify the calcium arc based on the degree of circumferential involvement (eg, ≤90° for single-quadrant involvement, 91°-180° for 2-quadrant involvement, etc.). These morphologic features have been shown to be predictive of short- and long-term response to treatment; in the population of 130 patients with symptomatic superficial femoral artery (SFA) disease included in the CODE study, Fujihara et al demonstrated that calcific lesions experienced significantly less lumen gain post intervention than noncalcified lesions.18 Calcium arcs ≥180° were also associated with worse lumen gain and higher rates of stent malapposition and restenosis post intervention.
Finally, when compared with angiography, IVUS has also been demonstrated to have superior ability in identifying thrombus in the infrainguinal vessels. In a small study that included 16 patients with infrainguinal PAD in the DETHROMBOSIS registry, Shammas et al demonstrated that IVUS was able to identify thrombus with definite certainty in 94.1% of patients in the registry, while angiography only identified definite thrombus (eg, TIMI thrombus grade 3) in 11.8% of cases.19 Notably, 58% of cases with evidence of thrombus on IVUS had no evidence of thrombus on angiography.
Intraprocedural
Intraprocedurally, existing literature suggests that IVUS use facilitates more appropriate balloon or stent sizing, which has the potential to impact outcomes post intervention. In the femoropopliteal vessels, this was demonstrated by Allan et al in a randomized controlled trial that assigned patients to either angiography alone or IVUS plus angiography and found that drug-coated balloon (DCB) diameters were significantly larger when IVUS was utilized.20 The authors hypothesized that this was the reason why patients in the IVUS-guided arm treated with DCB had significantly lower rates of restenosis post intervention (9% vs 38%, P=.001). In the infrapopliteal vessels, Fujihara et al demonstrated using a cohort of 216 patients with infrapopliteal chronic limb-threatening ischemia (CLTI) undergoing percutaneous transluminal angioplasty (PTA) that significantly larger balloon sizes were chosen during IVUS-guided procedures when compared with angiography-guided procedures (mean 2.5 ± 0.4 mm vs 2.2 ± 0.4 mm, P<.001).21 Patients in the IVUS-guided group were noted to experience significantly better skin perfusion pressures as well as better wound healing rates post intervention. Soga et al demonstrated similar findings in a retrospective study of 155 patients with infrapopliteal CLTI undergoing PTA, showing that IVUS-guided procedures used significantly larger balloons than angiography-guided procedures (3.1 ± 0.5 mm vs 2.6 ± 0.4 mm, P<.001).22 This translated into wound healing times that were 54 days shorter in the IVUS-guided group as well as higher rates of limb salvage without reintervention.
Several studies suggest that IVUS may also have utility in infrainguinal chronic total occlusion (CTO) intervention. In a retrospective cohort study of 44 patients (50 limbs) with infrainguinal CTOs, Takahashi et al demonstrated 96% technical success rates (full recanalization with TIMI 3 flow) and sustained freedom from 1-year target lesion revascularization of 78% when IVUS-imaging from a parallel vein was utilized to facilitate guidewire crossing.23 Baker et al demonstrated similar technical success rates of 90% and 1-year primary patency rates of 62% when IVUS-guided re-entry devices were utilized in a population of patients with lower extremity CTOs, 25% of which were infrainguinal.24 Both studies show promise for the use of IVUS in aiding successful CTO intervention and providing lasting outcomes for patients with infrainguinal PAD.
Postintervention
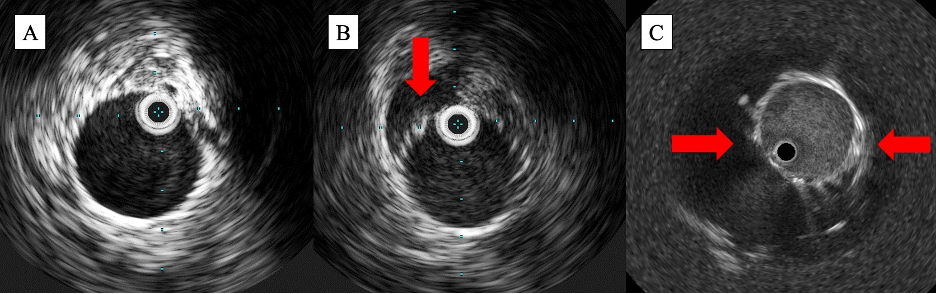
In the immediate postprocedural setting, IVUS is helpful in identifying complications including stent malapposition, stent underexpansion, and dissection (both stent- and non-stent-related). On IVUS, malapposition can be visualized when the echogenic strut of the stent fails to abut the vessel wall, with blood speckle visualizable between the 2 structures (Figures 3B and 3C). Stent underexpansion can be identified by measuring the minimum stent diameter and comparing that to the reference lumen diameter; in the coronary circulation, this is the strongest predictor of stent thrombosis and restenosis post intervention.25 Dissection can be identified by a break in the continuity of the vessel wall, with severity estimated based on the depth of dissection (intimal, medial, or extra-medial/adventitial), presence of blood speckle in the vessel wall (hematoma), and dissection length. While dissection can be visualized on angiography, several studies have demonstrated that IVUS has a superior ability to identify dissection in the infrainguinal vessels. In the iDissection studies, which included 15 patients with femoropopliteal PAD and 20 patients with infrapopliteal PAD, Shammas et al demonstrated that IVUS uncovered 5.8 times and 3.8 times more dissections after atherectomy than angiography in each vessel territory, respectively.26,27 These complications, when identified, can be addressed prior to the end of the case with either postdilation with a noncompliant balloon or additional stenting, facilitating better, more durable outcomes post intervention.
IVUS also has demonstrated utility in measuring response to an intervention. In the JETSTREAM Calcium Study, Maehara et al utilized IVUS to quantify both calcium removal (decrease in calcium area or arc of calcification) and luminal gain (change in minimum lumen area [MLA]) after rotational atherectomy in the femoropopliteal vessels.14 In the TRUE and TRUTH studies, Babaev et al and Singh et al similarly used IVUS and virtual histology IVUS to measure change in MLA and plaque volume after treatment with aspiration atherectomy and orbital atherectomy, respectively.15,16
Optical Coherence Tomography
In contrast to IVUS, which relies on the emission of acoustic signals, optical coherence tomography (OCT) functions through the emission of low-coherence, near-infrared light. These light waves reflect or scatter differentially based on the composition of a tissue and, when captured by an interferometer, can be used to generate a high-resolution cross-sectional image of a vessel.28 While there is substantially less data supporting its use in the infrainguinal circulation when compared with IVUS, emerging data suggest that it may have some utility as an adjunctive imaging modality in infrainguinal endovascular intervention. The following section describes the applications of OCT pre-, intra-, and post-infrainguinal intervention and explores situations where OCT use may confer benefits over IVUS during an intervention.
Preintervention
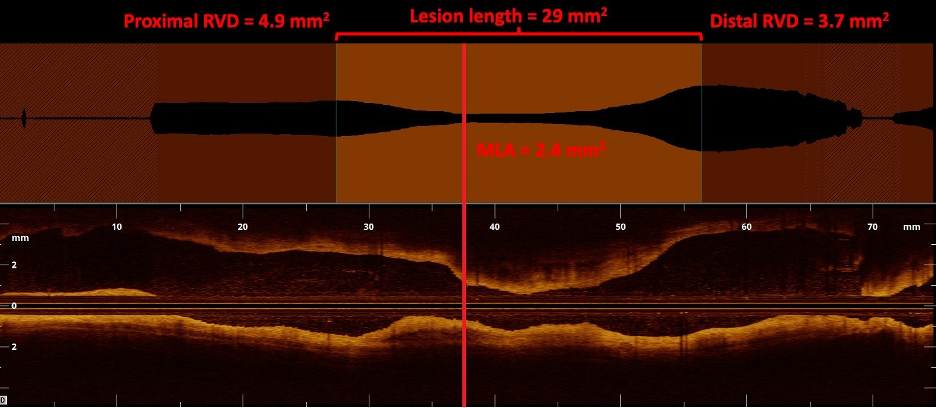
Preintervention, OCT can also be utilized to generate precise images of the target vessel and its components. When compared with IVUS, OCT offers up to 10 times greater spatial resolution, with axial and lateral resolutions of up to 10 µm and 25 µm, respectively.29,30 This enables more precise visualization of the layers of the vessel wall and demarcation of the borders of the vessel lumen. In a study led by Eberhardt et al comparing IVUS and OCT images of 112 popliteal and infrapopliteal arterial segments, blinded reviewers found OCT provided statistically greater vessel wall discriminability than IVUS.31 With this information, operators can make precise measurements of the MLA, RVD, and lesion length (Figure 4). Notably, this difference in vessel wall discriminability did not translate into a significant difference in luminal measurements.31 The improvement in spatial resolution also comes at the expense of penetration depth; due to the scattering and absorption of light waves by biological tissue, the penetration depth of OCT only reaches a maximum of 2 mm to 3 mm, much shallower than the 10 mm penetration depth provided with IVUS.29,30
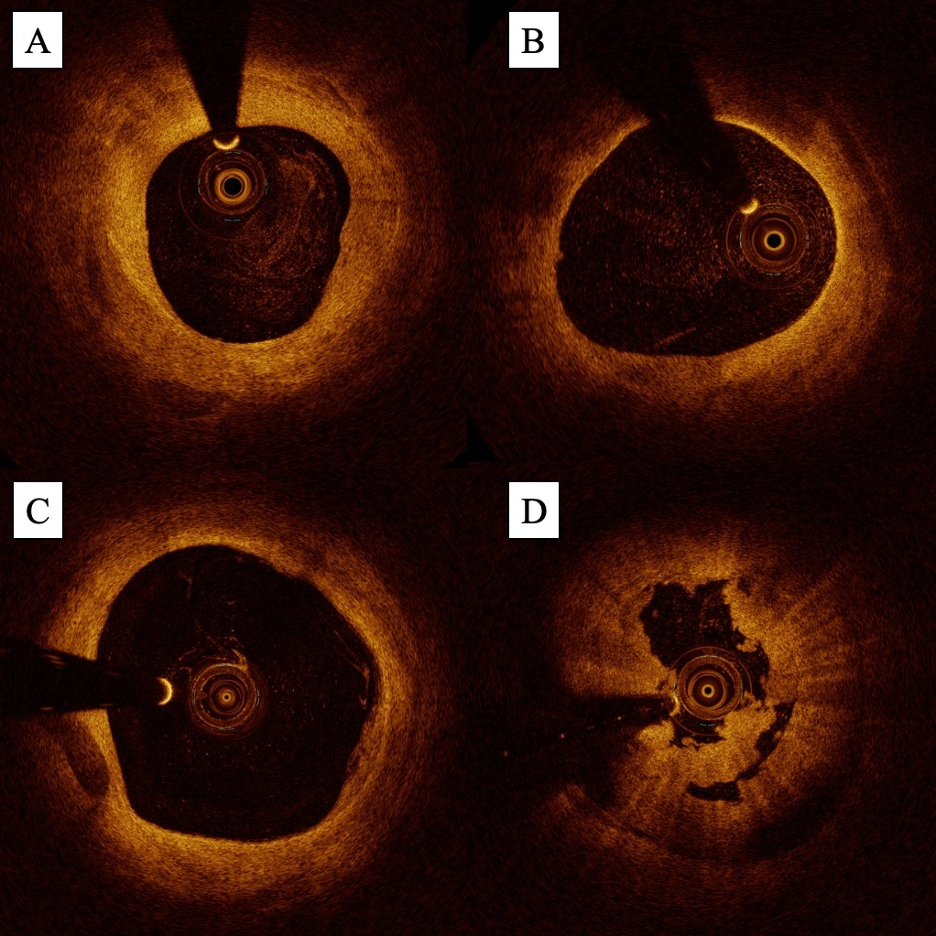
Similar to IVUS, OCT also enables detailed characterization of plaque composition. On OCT, fibrotic plaques appear bright (high signal) with low-signal attenuation (deep penetration of light), while lipid-rich plaques appear dark (low signal) with high signal attenuation (poor penetration of light) (Figures 5A and 5B). Calcified plaques also appear dark but can be differentiated by their low signal attenuation given that light is able to penetrate calcium (Figure 5C).32 Several small studies suggest that the higher image resolution of OCT allows it to characterize plaque better than IVUS;31,33 in a population of 12 patients with SFA PAD, Pavillard et al demonstrated that blinded operators rated both plaque characterization and calcium identification significantly better with OCT than IVUS.33 Bastante et al also demonstrated that OCT can be useful in helping operators determine if plaque modification is necessary prior to angioplasty or stenting.34
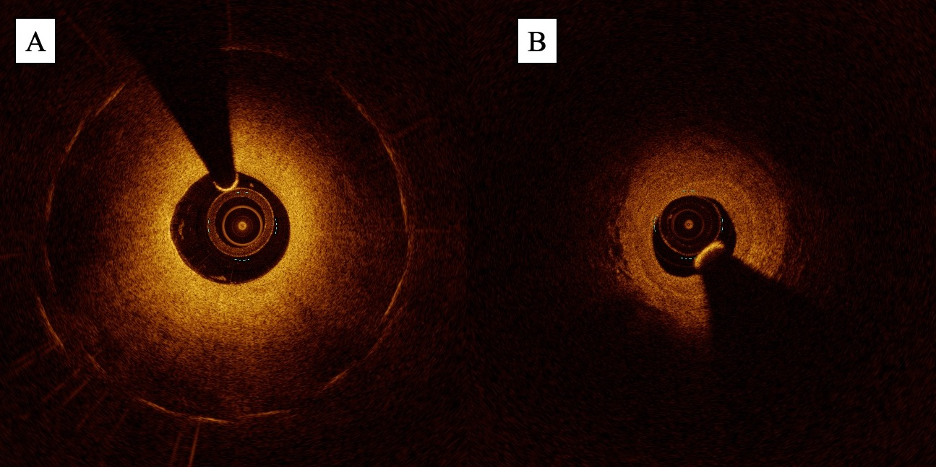
In the infrainguinal circulation, OCT has also demonstrated utility in identifying mechanisms of in-stent restenosis (ISR). While angiography can provide a generalized quantification of the extent of ISR (focal, edge-related, or diffuse), OCT allows for more granular evaluation of neointimal features including the presence of neointimal hyperplasia, neoatherosclerosis, and neovascularization (Figures 6A and 6B). Paraskevopoulos et al were the first to demonstrate the ability of OCT to identify these features in the infrainguinal circulation using a population of 12 patients with infrapopliteal PAD previously treated with drug-eluting stents (DES).35 The identification of these features may be of prognostic importance; in the coronary circulation, certain neointimal characteristics (eg, the presence of neoatherosclerosis) have been linked to higher rates of major adverse cardiac events.36,37
Similar to IVUS, OCT can also be utilized to identify thrombus in the infrainguinal vessels. On OCT, thrombi appear as bright (high signal) protrusions into the vessel lumen. Red and white thrombi can be differentiated based on the level of backscattering; red thrombi have high backscattering due to low light penetration and white thrombi have low backscattering (Figure 5D).31,38 In 2011, Karnabaditis et al demonstrated the ability of OCT to identify thrombus in the infrainguinal circulation using 27 femoropopliteal vessels.39 Following this, Yamamoto et al described the utility of OCT to guide thrombectomy during a case of acute limb ischemia, and Yang et al used OCT to characterize thrombus burden following post-stent implantation management with single- vs dual-antiplatelet therapy.40,41 While no literature exists to the authors’ knowledge comparing the sensitivity and specificity of thrombus identification in the infrainguinal circulation between OCT and IVUS, studies in the coronary circulation suggest that OCT may be superior for this use.42
OCT use has a considerable advantage over IVUS when it comes to the speed of image acquisition. While older-generation time-domain OCT devices relied on single-frequency light wave emissions and required proximal balloon occlusion to clear the vessel for imaging, current-generation Fourier-domain OCT devices can emit multiple frequencies simultaneously and utilize flush mediums as opposed to balloon occlusion to clear the vessel.30 This results in the generation of substantially higher frame rates (100 fps vs 30 fps in IVUS) along with pullback speeds that are 20 times faster than IVUS (20 mm/sec vs 1 mm/sec).30 With this speed of image generation, operators can image longer portions of a vessel in a substantially shorter period of time. Unfortunately, the technical requirements of OCT are also responsible for many of its limitations. Given that blood strongly scatters light, imaging artifacts can be generated if blood is not completely purged from the vessel. Iodinated contrast is most frequently utilized as the flush medium which, while allowing simultaneous recording of the angiogram, also carries the risk of contrast-induced kidney injury. While certain authors have demonstrated success with alternative flush mediums including dextran and heparinized saline, iodinated contrast remains the preferred medium for many operators.39,43
Intraprocedural
Several studies have demonstrated that OCT has utility for image acquisition during infrainguinal CTO intervention. Most of the research on intraprocedural OCT use has focused on the development and efficacy of the Ocelot system (Avinger), which uses real-time OCT imaging to visualize the catheter tip as it progresses through the CTO during recanalization.44 Initial success with this device was described in Europe by Schwindt et al, who demonstrated 94% technical success rates and 0% major adverse event (MAE) rates in a population of 33 patients with SFA CTOs.45 The use of OCT imaging resulted in significantly less contrast usage and shorter procedural times as compared with non-OCT-guided interventions. In the United States, CONNECT II, a prospective, nonrandomized, single-arm trial evaluating the Ocelot system, demonstrated a MAE rate of only 2% and a technical success rate of 72% alone and 97% if additional assist devices or re-entry devices were utilized in a population of 100 patients with mostly SFA CTOs.46 These findings were supported by a large prospective registry of 84 patients with femoropopliteal CTOs treated with the Ocelot device published by Schaefers et al, which demonstrated similar technical success rates of 73% as well as 3-year primary and secondary patency rates of 54.2% and 68.1%, respectively.47
OCT has also been utilized for image acquisition during atherectomy. The Pantheris atherectomy system (Avinger) incorporates an OCT laser aperture just proximal to the cutting edge of the atherectomy device, allowing for real-time visualization of a plaque during debulking. The VISION trial was the first large single-arm study investigating the Pantheris system in a population of 158 patients with femoropopliteal PAD and found technical success rates (reduction in the diameter of stenosis ≤50%) of 97% with 6-month MAE rates of 16.6%.48 Notably, 82% of the samples demonstrated sparing of the adventitia on histologic analysis, suggesting that OCT guidance enabled improved accuracy of plaque excision during atherectomy. Brodmann et al and Stavroulakis et al both described similar success rates as well as durable results in case series of 28 and 33 patients with lower extremity PAD treated with the Pantheris system, with primary patency rates ranging from 87% to 93% up to 15 months following the intervention.49,50
Postintervention
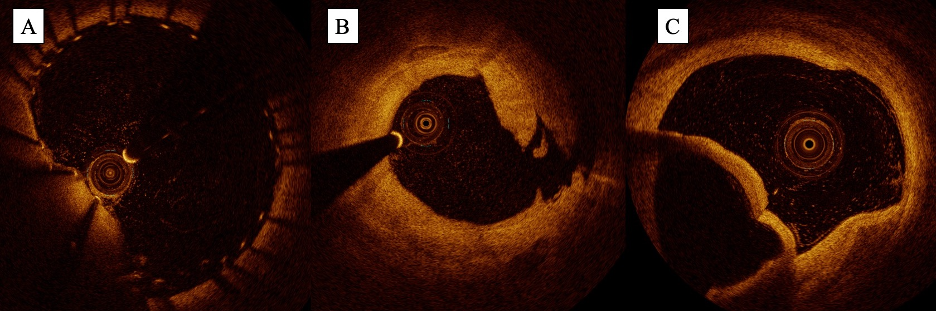
Following infrainguinal intervention, OCT has also demonstrated utility in identifying postprocedural complications including stent malapposition, plaque and tissue prolapse, and dissection. With the image resolution of OCT, the operator can clearly visualize the stent strut, enabling the identification of segments that do not abut the vessel wall (Figure 7A). Segments of the stent that have plaque or tissue protruding through the stent cells can also be visualized (eg, plaque or tissue prolapse). In the infrainguinal circulation, de Donato et al demonstrated examples of how OCT can identify stent malapposition and plaque prolapse in a case series of 15 patients with femoropopliteal PAD treated with endovascular intervention.51 Given its ability to visualize the vessel wall and its layers, OCT can also readily identify the presence and severity of dissection post intervention (Figure 7B and 7C); in a case series of 20 patients undergoing infrainguinal angioplasty, Karnabaditis et al identified dissections in 25% of cases after angioplasty using OCT, 60% of which were not visualizable by angiography.39 Identification of these dissections is crucial as it may change management prior to the end of the case. This is exemplified in a case report of SFA angioplasty published by Stefano et al in which a spiral dissection was identified by OCT when not visualizable on angiography, prompting additional stenting prior to the end of the case.52
Similar to IVUS, OCT has also been utilized to measure response to an intervention. In the DIAMOCT-PAD study, Cilingiroglu et al used OCT to characterize the immediate and long-term effects of orbital atherectomy in 7 patients with SFA PAD, demonstrating successful removal of atherosclerotic plaque and fibrous tissue and lasting improvements in luminal volume in the majority of patients.53 In the PRELUDE and PRELUDE BTK studies, Holden et al used OCT to demonstrate the efficacy of the Serranator angioplasty balloon (Cagent Vascular) in patients with femoropopliteal and infrapopliteal PAD, confirming the presence of serrations in all lesions and quantifying reductions in diameter stenosis.54,55 OCT has also been utilized by investigators to characterize vessel response to paclitaxel-coated balloons, paclitaxel-eluting stents, and intravascular lithotripsy both immediately after intervention and at 6-month follow-up.56-60
Summary, Takeaways, and Future Directions
Overall, this review demonstrates the many applications of IVUS and OCT in infrainguinal intervention, ranging from preintervention characterization of plaque morphology to postintervention assessment of response to treatment. Currently, of the 2 intravascular imaging modalities, IVUS has the strongest body of evidence supporting its use. This is reflected by recent consensus statements and by increasing rates of uptake across the United States.3,61
While it is well demonstrated to be safe and effective to use in the lower extremities, additional prospective data are needed to solidify the impact that IVUS has on rates of restenosis, revascularization, and amputation and to propel future use across the country. Compared with IVUS, the data supporting OCT use in the lower extremity is less robust, but safety and feasibility have been well demonstrated. Given its superior image quality, OCT may be favored over IVUS for specific indications, particularly in the setting of suspected vascular injury or stent-related complications (eg, stent-edge dissection, malapposition, ISR, etc.). n
The authors have completed and returned the ICMJE Form for Disclosure of Potential Conflicts of Interest. The authors report no financial relationships or conflicts of interest regarding the content herein.
Manuscript accepted May 11, 2023.
Address for correspondence: Sahil A. Parikh, MD, FACC, FSCAI, Associate Professor of Medicine, Columbia University Irving Medical Center and Columbia University Vagelos College of Physicians and Surgeons, 161 Fort Washington Ave., 6th Floor, New York, NY, 10032. Email: sap2196@cumc.columbia.edu
REFERENCES
1. Criqui MH, Matsushita K, Aboyans V, et al. Lower extremity peripheral artery disease: contemporary epidemiology, management gaps, and future directions: a scientific statement from The American Heart Association. Circulation. 2021;144(8): e171-e191. doi:10.1161/CIR.0000000000001005
2. Hirsch AT, Criqui MH, Treat-Jacobson D, et al. Peripheral arterial disease detection, awareness, and treatment in primary care. JAMA. 2001;286(11):1317-1324. doi:10.1001/jama.286.11.1317
3. Divakaran S, Parikh SA, Hawkins BM, et al. Temporal trends, practice variation, and associated outcomes with IVUS use during peripheral arterial intervention. JACC Cardiovasc Interv. 2022;15(20):2080-2090. doi:10.1016/j.jcin.2022.07.050
4. Gupta A, Shrivastava A, Vijayvergiya R, et al. Optical coherence tomography: an eye into the coronary artery. Front Cardiovasc Med. 2022;9:954554. doi:10.3389/fcvm.2022.854554
5. Mentias A, Sarrazin MV, Saad M, et al. Long-term outcomes of coronary stenting with and without use of intravascular ultrasound. JACC Cardiovasc Interv. 2020;13(16):1880-1890. doi:10.1016/j.jcin.2020.04.052
6. Ahn JM, Kang SJ, Yoon SH, et al. Meta-analysis of outcomes after intravascular ultrasound-guided versus angiography-guided drug-eluting stent implantation in 26,503 patients enrolled in three randomized trials and 14 observational studies. Am J Cardiol. 2014;113(8):1338-1347. doi:10.1016/j.amjcard.2013.12.043
7. Secemsky EA, Mosarla RC, Rosenfield K, et al. Appropriate use of intravascular ultrasound during arterial and venous lower extremity interventions. JACC Cardiovasc Interv. 2022;15(15): 1558-1568. doi:10.1016/j.jcin.2022.04.034
8. Iida O, Takahara M, Soga Y, et al. Vessel diameter evaluated by intravascular ultrasound versus angiography. J Endovasc Ther. 2022;29(3):343-349. doi:10.1177/15266028211047946
9. Pliagas G, Saab F, Stavroulakis K, et al. Intravascular ultrasound imaging versus digital subtraction angiography in patients with peripheral vascular disease. J Invasive Cardiol. 2020;32(3):99-103.
10. Shammas NW, Shammas WJ, Jones-Miller S, et al. Optimal vessel sizing and understanding dissections in infrapopliteal interventions: data from the iDissection Below the Knee study. J Endovasc Ther. 2020;27(4):575-580. doi:10.1177/1526602820924815
11. Kuku KO, Garcia-Garcia HM, Finizio M, et al. Comparison of angiographic and intravascular ultrasound vessel measurements in infra-popliteal endovascular interventions: the Below-the-Knee Calibration study. Cardiovasc Revasc Med. 2022;35:35-41. doi:10.1016/j.carrev.2021.09.004
12. Nissen SE, Yock P. Intravascular ultrasound: novel pathophysiological insights and current clinical applications. Circulation. 2001;103(4):604-616. doi:10.1161/01.cir.103.4.604
13. Yin D, Maehara A, Shimshak TM, et al. Intravascular ultrasound validation of contemporary angiographic scores evaluating the severity of calcification in peripheral arteries. J Endovasc Ther. 2017;24(4):478-487. doi:101177/1526602817708796
14. Maehara A, Mintz GS, Shimshak TM, et al. Intravascular ultrasound evaluation of JETSTREAM atherectomy removal of superficial calcium in peripheral arteries. EuroIntervention. 2015;11(1):96-103. doi:10.4244/EIJV11I1A17
15. Singh T, Koul D, Szpunar S, et al. Tissue Removal by ultrasound evaluation (the TRUE study): the Jetstream G2 system post-market peripheral vascular IVUS study. J Invasive Cardiol. 2011;23(7):269-273.
16. Babaev A, Zavlunova S, Attubato MJ, et al. Orbital atherectomy plaque modification assessment of the femoropopliteal artery via intravascular ultrasound (TRUTH study). Vasc Endovascular Surg. 2015;49(7):188-194. doi:10.1177/1538574415607361
17. Tielbeek AV, Vroegindeweij D, Buth J, Schol FP, Mali WP. Comparison of intravascular ultrasonography and intraarterial digital subtraction angiography after directional atherectomy of short lesions in femoropopliteal arteries. J Vasc Surg. 1996;23(3):436-445. doi:10.1016/s0741-5214(96)80008-5
18. Fujihara M, Kozuki A, Tsubakimoto Y, et al. lumen gain after endovascular therapy in calcified superficial femoral artery occlusive disease assessed by intravascular ultrasound (CODE study). J Endovasc Ther. 2019;26(3):322-330. doi:10.1177/1526602819836095
19. Shammas NW, Dippel EJ, Shammas G, Gayton L, Coiner D, Jerin M. Dethrombosis of the lower extremity arteries using the power-pulse spray technique in patients with recent onset thrombotic occlusions: results of the DETHROMBOSIS registry. J Endovasc Ther. 2008;15(5):570-579. doi:10.1583/08-2453.1
20. Allan RB, Puckridge PJ, Spark JI, Delaney CL. The impact of intravascular ultrasound on femoropopliteal artery endovascular interventions: a randomized controlled trial. JACC Cardiovasc Interv. 2022;15(5):536-546. doi:10.1016/j.jcin.2022.01.001
21. Fujihara M, Yazu Y, Takahara M. intravascular ultrasound-guided interventions for below-the-knee disease in patients with chronic limb-threatening ischemia. J Endovasc Ther. 2020;27(4):565-574. doi:10.1177/1526602820935606
22. Soga Y, Takahara M, Ito N, et al. Clinical impact of intravascular ultrasound-guided balloon angioplasty in patients with chronic limb threatening ischemia for isolated infrapopliteal lesion. Catheter Cardiovasc Interv. 2021;97(3):E376-E384. doi:10.1002/ccd.29347
23. Takahashi Y, Sato T, Okazaki H, et al. Transvenous intravascular ultrasound-guided endovascular treatment for chronic total occlusion of the infrainguinal arteries. J Endovasc Ther. 2017;24(5):718-726. doi:10.1177/1526602817723139
24. Baker AC, Humphries MD, Noll RE Jr, et al. Technical and early outcomes using ultrasound-guided reentry for chronic total occlusions. Ann Vasc Surg. 2015;29(1):55-62. doi:10.1016/j.avsg.2014.10.011
25. Fujii K, Carlier SG, Mintz GS, et al. Stent underexpansion and residual reference segment stenosis are related to stent thrombosis after sirolimus-eluting stent implantation: an intravascular ultrasound study. J Am Coll Cardiol. 2005;45(7):995-998. doi:10.1016/j.jacc.2004.12.066
26. Shammas NW, Tory JT, Shammas WJ, Jones-Miller S, Shammas GA. Intravascular ultrasound assessment and correlation with angiographic findings demonstrating femoropopliteal arterial dissections post atherectomy: results from the iDissection study. J Invasive Cardiol. 2018;30(7):240-244.
27. Shammas NW, Shammas WJ, Jones-Miller S, et al. Optimal vessel sizing and understanding dissections in infrapopliteal interventions: data from the iDissection below the knee study. J Endovasc Ther. 2020;27(4):575-580. doi:10.1177/1526602820924815
28. Marschall S, Sander B, Mogensen M, Jørgensen TM, Andersen PE. Optical coherence tomography-current technology and applications in clinical and biomedical research. Anal Bioanal Chem. 2011;400(9):2699-2720. doi:10.1007/s00216-011-5008-1
29. Secco GG, Grattoni C, Parisi R, et al. Optical coherence tomography guidance during peripheral vascular intervention. Cardiovasc Intervent Radiol. 2015;38(3):768-772. doi:10.1007/s00270-014-0868-3
30. Negi SI, Rosales O. The role of intravascular optical coherence tomography in peripheral percutaneous interventions. J Invasive Cardiol. 2013;25(3):E51-E53.
31. Eberhardt KM, Treitl M, Boesenecker K, Maxien D, Reiser M, Rieger J. Prospective evaluation of optical coherence tomography in lower limb arteries compared with intravascular ultrasound. J Vasc Interv Radiol. 2013;24(10):1499-1508. doi:10.1016/j.jvir.2013.06.015
32. Bezerra HG, Costa MA, Guagliumi G, Rollins AM, Simon DI. Intracoronary optical coherence tomography: a comprehensive review clinical and research applications. JACC Cardiovasc Interv. 2009;2(11):1035-1046. doi:10.1016/j.jcin.2009.06.019
33. Pavillard E, Sewall L. A post-market, multi-vessel evaluation of the imaging of peripheral arteries for diagnostic purposeS comparing optical Coherence tomogrApy and iNtravascular ultrasound imaging (SCAN). BMC Med Imaging. 2020;20(1):18. doi:10.1186/S12880-020-0420-7
34. Bastante T, Rivero F, Cuesta J, Alfonso F. Calcified neoatherosclerosis causing “undilatable” in-stent restenosis: insights of optical coherence tomography and role of rotational atherectomy. JACC Cardiovasc Interv. 2015;8(15):2039-2040. doi:10.1016/j.jcin.2015.08.024
35. Paraskevopoulos I, Spiliopoulos S, Davlouros P, et al. Evaluation of below-the-knee drug-eluting stents with frequency-domain optical coherence tomography: neointimal hyperplasia and neoatherosclerosis. J Endovasc Ther. 2013;20(1):80-93. doi:10.1583/12-4091.1
36. Kim JS, Lee JH, Shin DH, et al. Long-term outcomes of neointimal hyperplasia without neoatherosclerosis after drug-eluting stent implantation. JACC Cardiovasc Imaging. 2014;7(8):788-795. doi:10.1016/j.jcmg.2014.05.004
37. Sakakura K, Joner M, Virmani R. Does neointimal characterization following DES implantation predict long-term outcomes? JACC Cardiovasc Imaging. 2014;7(8):796-798. doi:10.1016/j.jcmg.2014.06.002
38. Kume T, Akasaka T, Kawamoto T, et al. Assessment of coronary arterial thrombus by optical coherence tomography. Am J Cardiol. 2006;97(12):1713-1717. doi:10.1016/j.amjcard.2006.01.03
39. Karnabatidis D, Katsanos K, Paraskevopoulos I, Diamantopoulos A, Spiliopoilos S, Siablis D. Frequency-domain intravascular optical coherence tomography of the femoropopliteal artery. Cardiovasc Intervent Radiol. 2011;34(6):1172-1181. doi:10.1007/s00270-010-0092-8
40. Yamamoto Y, Kawarada O, Sakamoto S, et al. Progression of intimal hyperplasia and multiple-channel formation after Fogarty thrombectomy: insight into vasculopathy from optical coherence tomography and intravascular ultrasound findings. JACC Cardiovasc Interv. 2015;8(15):e251-e253. doi:10.1016/j.jcin.2015.07.036
41. Yang X, Leesar MA, Ahmed H, et al. Impact of ticagrelor and aspirin versus clopidogrel and aspirin in symptomatic patients with peripheral arterial disease: thrombus burden assessed by optical coherence tomography. Cardiovasc Revasc Med. 2018;19(7 Pt A):778-784. doi:10.1016/j.carrev.2018.02.013
42. Kubo T, Imanishi T, Takarada S, et al. Assessment of culprit lesion morphology in acute myocardial infarction: ability of optical coherence tomography compared with intravascular ultrasound and coronary angioscopy. J Am Coll Cardiol. 2007;50(10):933-939. doi:10.1016/j.jacc.2007.04.082
43. Kendrick DE, Allemang MT, Gosling AF, et al. Dextran or saline can replace contrast for intravascular optical coherence tomography in lower extremity arteries. J Endovasc Ther. 2016;23(5):723-730. doi:10.1177/1526602816657392
44. Marmagkiolis K, Lendel V, Cawich I, Cilingiroglu M. Ocelot catheter for the recanalization of lower extremity arterial chronic total occlusion. Cardiovasc Revasc Med. 2014;15(1):46-49. doi:10.1016/j.carrev.2013.09.007
45. Schwindt A, Reimers B, Scheinert D, et al. Crossing chronic total occlusions with the Ocelot system: The initial European experience. EuroIntervention. 2013;9(7):854-862. doi:10.4244/EIJV9I7A139
46. Selmon MR, Schwindt AG, Cawich IM, et al. Final results of the Chronic Total Occlusion Crossing With the Ocelot System II (CONNECT II) study. J Endovasc Ther. 2013;20(6):770-781. doi:10.1583/13-4380MR.1
47. Schaefers JF, Schwindt AG, Maritati G, Torsello G, Pannucio G. Outcome after crossing femoropopliteal chronic total occlusions based on optical coherence tomography guidance. Vasc Endovascular Surg. 2018;52(1):27-33. doi:10.1177/1538574417740057
48. Schwindt AG, Bennett JG Jr, Crowder WH, et al. Lower extremity revascularization using optical coherence tomography-guided directional atherectomy: final results of the EValuatIon of the PantheriS OptIcal COherence Tomography ImagiNg atherectomy system for use in the peripheral vasculature (VISION) study. J Endovasc Ther. 2017;24(3):355-366. doi:10.1177/1526602817701720
49. Stavroulakis K, Bisdas T, Torsello G, Argyriou A, Bollenberg L, Schwindt A. Optical coherence tomography guided directional atherectomy with antirestenotic therapy for femoropopliteal arterial disease. J Cardiovasc Surg (Torino). 2019;60(2):191-197. doi:10.23736/S0021-9509.19.10843-9
50. Brodmann M. TCT-786 6-month duplex patency and MRA following OCT guided atherectomy: single center prospective case series. J Am Coll Cardiol. 2016;68(18S):B318. doi:10.1016/j.jacc.2016.09.817
51. de Donato G, Setacci F, Galzerano G, Borrelli MP, Ruzzi U, Setacci C. PS80. Safety and feasibility of intravascular optical coherence tomography to evaluate lesions of femoropopliteal arterial segment before and after endovascular treatment. J Vasc Surg. 2014;59(6):54S. doi:10.1016/j.jvs.2014.03.121
52. Stefano GT, Mehanna E, Parikh SA. Imaging a spiral dissection of the superficial femoral artery in high resolution with optical coherence tomography—seeing is believing. Catheter Cardiovasc Interv. 2013;81(3):568-572. doi:10.1002/ccd.24292
53. Cilingiroglu M, Kilic ID, Hoyt T, et al. DIAMondback Atherectomy With OCT Visualization for Calcified PAD Lesions (DIAMOCT-PAD study). J Invasive Cardiol. 2022;34(2):E117-E123.
54. Holden A, Hill A, Walker A, et al. PRELUDE prospective study of the Serranator device in the treatment of atherosclerotic lesions in the superficial femoral and popliteal arteries. J Endovasc Ther. 2019;26(1):18-25. doi:10.1177/1526602818820787
55. Holden A, Lichtenberg M, Nowakowski P, et al. Prospective study of serration angioplasty in the infrapopliteal arteries using the Serranator device: PRELUDE BTK Study. J Endovasc Ther. 2022;29(4):586-593. doi:10.1177/15266028211059917
56. Kozuki A, Shinke T, Otake H, et al. Optical coherence tomography study of chronic-phase vessel healing after implantation of bare metal and paclitaxel-eluting self-expanding nitinol stents in the superficial femoral artery. J Cardiol. 2016;67(5):424-429. doi:10.1016/j.jjcc.2015.06.011
57. Hoyt T, Feldman MD, Okutucu S, et al. Assessment of vascular patency and inflammation with intravascular optical coherence tomography in patients with superficial femoral artery disease treated with Zilver PTX stents. Cardiovasc Revasc Med. 2020;21(1):101-107. doi:10.1016/j.carrev.2019.07.009
58. Virmani R, Finn AV, Kutyna M, et al. Pulsatile intravascular lithotripsy: a novel mechanism for peripheral artery calcium fragmentation and luminal expansion. Cardiovasc Revasc Med. 2023;50:43-53. doi:10.1016/J.CARREV.2023.01.003
59. Miki K, Fujii K, Shibuya M, et al. Impact of stent diameter on vascular response after self-expanding paclitaxel-eluting stent implantation in the superficial femoral artery. J Cardiol. 2017;70(4):346-352. doi: 10.1016/j.jjcc.2016.12.011
60. Chowdhury MM, Singh K, Albaghdadi MS, et al. Paclitaxel drug-coated balloon angioplasty suppresses progression and inflammation of experimental atherosclerosis in rabbits. JACC Basic Transl Sci. 2020;5(7):685-695. doi:10.1016/j.jacbts.2020.04.007
61. Secemsky EA, Mosarla RC, Rosenfield K, et al. Appropriate use of intravascular ultrasound during arterial and venous lower extremity interventions. JACC Cardiovasc Interv. 2022;15(15):1558-1568. doi:10.1016/j.jcin.2022.04.034